Atmospheric reentry

Atmospheric reentry is the process by which vehicles that are outside the atmosphere of a planet can enter that atmosphere and reach the planetary surface intact. Vehicles that undergo this process include spacecraft from orbit, as well as suborbital ballistic missile reentry vehicles. Typically this process requires special methods to protect against aerodynamic heating. Various advanced technologies have been developed to enable atmospheric reentry and flight at extreme velocities.
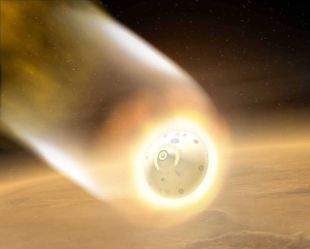
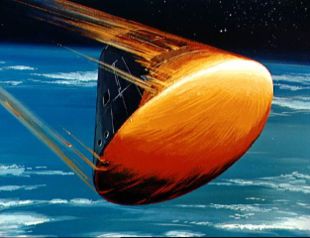
History
The technology of atmospheric reentry was a consequence of the Cold War. Ballistic missiles and nuclear weapons were legacies of World War II left to both the Soviet Union and the United States. Both nations initiated massive research and development programs to further the military capability of those technologies. However before a missile-delivered nuclear weapon could be practical there lacked an essential ingredient: an atmospheric reentry technology. In theory, the nation first developing reentry technology had a decisive military advantage, yet it was unclear whether the technology was physically possible. Basic calculations showed the kinetic energy of a nuclear warhead returning from orbit was sufficient to completely vaporize the warhead. Despite these calculations the military stakes were so high that simply assuming atmospheric reentry's impossibility was unacceptable, and it was known that meteorites were able to successfully reach ground level. Consequently a high-priority program was initiated to develop reentry technology. Atmospheric reentry was successfully developed, which made possible nuclear-armed intercontinental ballistic missiles.
The technology was further pushed forward for human use by another consequence of the Cold War. The Soviet Union saw a propaganda and military advantage in pursuing space exploration. To the embarrassment of the United States, the Soviet Union orbited an artificial satellite, followed by a series of other technological firsts that culminated with a Soviet cosmonaut orbiting the Earth and returning safely to Earth. Many of these achievements were enabled through atmospheric reentry technology. The United States saw the Soviet Union's achievements as a challenge to its national pride as well as a threat to national security. Consequently the United States followed the Soviet Union's initiative and increased its nascent Space Program thus beginning the Space Race.
Terminology, definitions and jargon
Over the decades since the 1950s, a rich technical jargon has grown around the engineering of vehicles designed to enter planetary atmospheres. It is recommended that the reader review the jargon glossary before continuing with this article on atmospheric reentry.
Blunt body entry vehicles
These four shadowgraph images represent early reentry-vehicle concepts. A shadowgraph is a process that makes visible the disturbances that occur in a fluid flow at high velocity, in which light passing through a flowing fluid is refracted by the density gradients in the fluid resulting in bright and dark areas on a screen placed behind the fluid.
H. Julian Allen and A. J. Eggers, Jr. of the National Advisory Committee for Aeronautics (NACA) made the counterintuitive discovery in 1952 that a blunt shape (high drag) made the most effective heat shield. From simple engineering principles, Allen and Eggers showed that the heat load experienced by an entry vehicle was inversely proportional to the drag coefficient, i.e. the greater the drag, the less the heat load. Through making the reentry vehicle blunt, the shock wave and heated shock layer were pushed forward, away from the vehicle's outer wall. Since most of the hot gases were not in direct contact with the vehicle, the heat energy would stay in the shocked gas and simply move around the vehicle to later dissipate into the atmosphere.
The Allen and Eggers discovery, though initially treated as a military secret, was eventually published in 1958.[1] The Blunt Body Theory made possible the heat shield designs that were embodied in the Mercury, Gemini and Apollo space capsules, enabling astronauts to survive the fiery reentry into Earth's atmosphere.
Entry vehicle shapes
There are several basic shapes used in designing entry vehicles:
Sphere or spherical section
The simplest axisymmetric shape is the sphere or spherical section. This can either be a complete sphere or a spherical section forebody with a converging conical afterbody. The sphere or spherical section's aerodynamics are easy to model analytically using Newtonian impact theory. Likewise, the spherical section's heat flux can be accurately modeled with the Fay-Riddell equation.[2] The static stability of a spherical section is assured if the vehicle's center-of-mass is upstream from the center-of-curvature (dynamic stability is more problematic). Pure spheres have no lift. However by flying at an angle-of-attack, a spherical section has modest aerodynamic lift thus providing some cross-range capability and widening its entry corridor. In the late 1950s and early 1960s, high speed computers were not yet available and CFD was still embryonic. Because the spherical section was susceptible to closed form analysis, that geometry became the default for conservative design. Consequently, manned capsules of that era were based upon the spherical section. Pure spherical entry vehicles were used in the early Soviet Vostok. The most famous example of a spherical section entry vehicle was the Apollo Command Module (Apollo-CM), using a spherical section forebody heatshield with a converging conical afterbody. The Apollo-CM (AS-501) flew a lifting-entry with a hypersonic trim angle-of-attack of −27° (0° is blunt-end first) to yield an average L/D of 0.368.[3] This angle-of-attack was achieved by precisely offsetting the vehicle's center-of-mass from its axis-of-symmetry. Other examples of the spherical section geometry as manned capsules are Soyuz/Zond, Gemini and Mercury.
Sphere-cone
The sphere-cone is a spherical section with a frustum attached. The sphere-cone's dynamic stability is typically better than that of a spherical section. With a sufficiently small half-angle and properly placed center-of-mass, a sphere-cone can provide aerodynamic stability from Keplerian entry to surface impact. The original American sphere-cone aeroshell was the Mk-2 RV which was developed in 1955 by the General Electric Corp. The Mk-2's design was derived from blunt body theory and used a radiatively cooled thermal protection system (TPS) based upon a metallic heat shield (the different TPS types are later described in this article). The Mk-2 had significant defects as a weapon delivery system, i.e. it loitered too long in the upper atmosphere due to its lower ballistic coefficient and also trailed a stream of vaporized metal making it very visible to radar. These defects made the Mk-2 overly susceptible to anti-ballistic missile (ABM) systems. Consequently an alternative sphere-cone RV to the Mk-2 was developed by General Electric.
This new RV was the Mk-6 which used a non-metallic ablative TPS (nylon phenolic). This new TPS was so effective as a reentry heat shield that significantly reduced bluntness was possible. However the Mk-6 was a huge RV with an entry mass of 3360 kg, a length of 3.1 meters and a half-angle of 12.5°. Subsequent advances in nuclear weapon and ablative TPS design allowed RVs to become significantly smaller with a further reduced bluntness ratio compared to the Mk-6. Since the 1960s, the sphere-cone has become the preferred geometry for modern ICBM RVs with typical half-angles being between 10° to 11°.
Reconnaissance satellite RVs (recovery vehicles) also used a sphere-cone shape and were the first American example of a non-munition entry vehicle (Discoverer-I, launched on 28 February 1959). The sphere-cone was later used for space exploration missions to other celestial bodies or for return from open space, e.g. Stardust probe. Unlike with military RVs, the advantage of the blunt body's lower TPS mass remained with space exploration entry vehicles like the Galileo Probe with a half angle of 45° or the Viking aeroshell with a half angle of 70°. Space exploration sphere-cone entry vehicles have landed on the surface or entered the atmospheres of Mars, Venus, Jupiter and Titan.
Biconic
The biconic is a sphere-cone with an additional frustum attached. The biconic offers a significantly improved L/D ratio. A biconic designed for Mars aerocapture typically has an L/D of approximately 1.0 compared to an L/D of 0.368 for the Apollo-CM. The higher L/D makes a biconic shape better suited for transporting people to Mars due to the lower peak deceleration. Arguably, the most significant biconic ever flown was the Advanced Maneuverable Reentry Vehicle (AMaRV). Four AMaRVs were made by the McDonnell-Douglas Corp. and represented a quantum leap in RV sophistication. Three of the AMaRVs were launched by Minuteman-1 ICBMs on 20 December 1979, 8 October 1980 and 4 October 1981. AMaRV had an entry mass of approximately 470 kg, a nose radius of 2.34 cm, a forward frustum half-angle of 10.4°, an inter-frustum radius of 14.6 cm, aft frustum half angle of 6°, and an axial length of 2.079 meters. No accurate diagram or picture of AMaRV has ever appeared in the open literature. However a schematic sketch of an AMaRV-like vehicle along with trajectory plots showing hairpin turns has been published.[4]
AMaRV's attitude was controlled through a split body flap (also called a "split-windward flap") along with two yaw flaps mounted on the vehicle's sides. Hydraulic actuation was used for controlling the flaps. AMaRV was guided by a fully autonomous navigation system designed for evading anti-ballistic missile (ABM) interception. The McDonnell Douglas DC-X (also a biconic) was essentially a scaled up version of AMaRV. AMaRV and the DC-X also served as the basis for an unsuccessful proposal for what eventually became the Lockheed Martin X-33. Amongst aerospace engineers, AMaRV has achieved legendary status along side such technological marvels as the SR-71 Blackbird and the N-1 rocket.
Non-axisymmetric shapes
Non-axisymmetric shapes have been used for manned entry vehicles. One example is the winged orbit vehicle that uses a delta wing for maneuvering during descent much like a conventional glider. This approach has been used by the American Space Shuttle and the Soviet Buran. The lifting body is another entry vehicle geometry and was used with the X-23 PRIME (Precision Recovery Including Maneuvering Entry) vehicle.
The FIRST (Fabrication of Inflatable Re-entry Structures for Test) system was an Aerojet proposal for an inflated-spar Rogallo wing made up from Inconel wire cloth impregnated with silicone rubber and Silicon Carbide dust. FIRST was proposed in both one-man and six man versions, used for emergency escape and reentry of stranded space station crews, and was based on an earlier unmanned test program that resulted in a partially successful reentry flight from space (the launcher nose cone fairing hung up on the material, dragging it too low and fast for the TPS, but otherwise it appears the concept would have worked, even with the fairing dragging it, the test article flew stably on reentry until burn-through).
The proposed MOOSE system would have used a one-man inflatable ballistic capsule as an emergency astronaut entry vehicle. This concept was carried further by the Douglas Paracone project. While these concepts were unusual, the inflated shape on reentry was in fact axisymmetric.
Shock layer gas physics
An approximate rule-of-thumb used by heat shield designers for estimating peak shock layer temperature is to assume the air temperature in Kelvin to be equal to the entry speed in meters per second. For example, a spacecraft entering the atmosphere at 7.8 km/s would experience a peak shock layer temperature of 7800 K. This method of estimation is a mathematical accident and a consequence of peak heat flux for terrestrial entry typically occurring around 60 km altitude.
It is clear that 7800 K is incredibly hot (the surface of the sun, or photosphere, is only 6000 K). For such high temperatures, the air in the shock layer will break down chemically (dissociate) and also become ionized. This chemical dissociation necessitates various physical models to describe the air's thermal and chemical properties. There are four basic physical models of a gas that are important to aeronautical engineers who design heat shields:
Perfect gas model
Almost all aeronautical engineers are taught the perfect (ideal) gas model during their undergraduate education. Most of the important perfect gas equations along with their corresponding tables and graphs are shown in NACA Report 1135.[5] Excerpts from NACA Report 1135 often appear in the appendices of thermodynamics textbooks and are familiar to most aeronautical engineers who design supersonic aircraft.
Perfect gas theory is elegant and extremely useful for designing aircraft but assumes the gas is chemically inert. From the standpoint of aircraft design, air can be assumed to be inert for temperatures less than 550 K at one atmosphere pressure. Perfect gas theory begins to break down at 550 K and is not usable at temperatures greater than 2000 K. For temperatures greater than 2000 K, a heat shield designer must use a real gas model.
Real (equilibrium) gas model
The real gas equilibrium model is normally taught to aeronautical engineers studying towards a master's degree. Not surprisingly, it is a common error for a bachelor's-level engineer to incorrectly use perfect-gas theory on a hypersonic design. An entry vehicle's pitching moment can be significantly influenced by real-gas effects. Both the Apollo-CM and the Space Shuttle were designed using incorrect pitching moments determined through inaccurate real-gas modeling. The Apollo-CM's trim-angle angle-of-attack was higher than originally estimated, resulting in a narrower lunar return entry corridor. The actual aerodynamic center of the Columbia was upstream from the calculated value due to real-gas effects. On Columbia’s maiden flight (STS-1), astronauts John W. Young and Robert Crippen had some anxious moments during reentry when there was concern about losing control of the vehicle.
An equilibrium real-gas model assumes that a gas is chemically reactive but also assumes all chemical reactions have had time to complete and all components of the gas have the same temperature (this is called thermodynamic equilibrium). When air is processed by a shock wave, it is superheated by compression and chemically dissociates through many different reactions (contrary to myth, friction is not the main cause of shock-layer heating). The distance from the shock wave to the stagnation point on the entry vehicle's leading edge is called shock wave stand off. An approximate rule of thumb for shock wave standoff distance is 0.14 times the nose radius. One can estimate the time of travel for a gas molecule from the shock wave to the stagnation point by assuming a free stream velocity of 7.8 km/s and a nose radius of 1 meter, i.e. time of travel is about 18 microseconds. This is roughly the time required for shock-wave-initiated chemical dissociation to approach chemical equilibrium in a shock layer for a 7.8 km/s entry into air during peak heat flux. Consequently, as air approaches the entry vehicle's stagnation point, the air effectively reaches chemical equilibrium thus enabling an equilibrium model to be usable. For this case, most of the shock layer between the shock wave and leading edge of an entry vehicle is chemically reacting and not in a state of equilibrium. The Fay-Riddell equation, which is of extreme importance towards modeling heat flux, owes its validity to the stagnation point being in chemical equilibrium. It should be emphasized that the time required for the shock layer gas to reach equilibrium is strongly dependent upon the shock layer's pressure. For example, in the case of the Galileo Probe's entry into Jupiter's atmosphere, the shock layer was mostly in equilibrium during peak heat flux due to the very high pressures experienced (this is counter intuitive given the free stream velocity was 39 km/s during peak heat flux) .
Determining the thermodynamic state of the stagnation point is more difficult under an equilibrium gas model than a perfect gas model. Under a perfect gas model, the ratio of specific heats (also called "isentropic exponent", "adiabatic index", "gamma" or "kappa") is assumed to be constant along with the gas constant. For a real gas, the ratio of specific heats can wildly oscillate as a function of temperature. Under a perfect gas model there is an elegant set of equations for determining thermodynamic state along a constant entropy stream line called the isentropic chain. For a real gas, the isentropic chain is unusable and a Mollier diagram would be used instead for manual calculation. However graphical solution with a Mollier diagram is now considered obsolete with modern heat shield designers using computer programs based upon a digital lookup table (another form of Mollier diagram) or a chemistry based thermodynamics program. The chemical composition of a gas in equilibrium with fixed pressure and temperature can be determined through the Gibbs free energy method. Gibbs free energy is simply the total enthalpy of the gas minus its total entropy times temperature. A chemical equilibrium program normally does not require chemical formulas or reaction rate equations. The program works by preserving the original elemental abundances specified for the gas and varying the different molecular combinations of the elements through numerical iteration until the lowest possible Gibbs free energy is calculated (a Newton-Raphson method is the usual numerical scheme). The data base for a Gibbs free energy program comes from spectroscopic data used in defining partition functions. Among the best equilibrium codes in existence is the program Chemical Equilibrium with Applications (CEA) which was written by Bonnie J. McBride and Sanford Gordon at NASA Lewis (now renamed "NASA Glenn Research Center"). Other names for CEA are the "Gordon and McBride Code" and the "Lewis Code". CEA is quite accurate up to 10,000 K for planetary atmospheric gases but unusable beyond 20,000 K (double ionization is not modeled). CEA can be downloaded from the Internet along with full documentation and will compile on Linux under the G77 Fortran compiler.
Real (non-equilibrium) gas model
A non-equilibrium real gas model is the most accurate model of a shock layer's gas physics but is more difficult to solve than an equilibrium model. The simplest non-equilibrium model is the Lighthill-Freeman model.[6][7] The Lighthill-Freeman model initially assumes a gas made up of a single diatomic species susceptible to only one chemical formula and its reverse, e.g. N2 → N + N and N + N → N2 (dissociation and recombination). Because of its simplicity, the Lighthill-Freeman model is a useful pedagogical tool but is unfortunately too simple for modeling non-equilibrium air. Air is typically assumed to have a mole fraction composition of 0.7812 molecular nitrogen, 0.2095 molecular oxygen and 0.0093 argon. The simplest real gas model for air is the five species model which is based upon N2, O2, NO, N and O. The five species model assumes no ionization and ignores trace species like carbon dioxide.
When running a Gibbs free energy equilibrium program, the iterative process from the originally specified molecular composition to the final calculated equilibrium composition is essentially random and not time accurate. With a non-equilibrium program, the computation process is time accurate and follows a solution path dictated by chemical and reaction rate formulas. The five species model has 17 chemical formulas (34 when counting reverse formulas). The Lighthill-Freeman model is based upon a single ordinary differential equation and one algebraic equation. The five species model is based upon 5 ordinary differential equations and 17 algebraic equations. Because the 5 ordinary differential equations are loosely coupled, the system is numerically "stiff" and difficult to solve. The five species model is only usable for entry from low Earth orbit where entry velocity is approximately 7.8 km/s. For lunar return entry of 11 km/s, the shock layer contains a significant amount of ionized nitrogen and oxygen. The five species model is no longer accurate and a twelve species model must be used instead. High speed Mars entry which involves a carbon dioxide, nitrogen and argon atmosphere is even more complex requiring a 19 species model.
An important aspect of modeling non-equilibrium real gas effects is radiative heat flux. If a vehicle is entering an atmosphere at very high speed (hyperbolic trajectory, lunar return) and has a large nose radius then radiative heat flux can dominate TPS heating. Radiative heat flux during entry into an air or carbon dioxide atmosphere typically comes from unsymmetric diatomic molecules, e.g. cyanogen (CN), carbon monoxide, nitric oxide (NO), single ionized molecular nitrogen, etc. These molecules are formed by the shock wave dissociating ambient atmospheric gas followed by recombination within the shock layer into new molecular species. The newly formed diatomic molecules initially have a very high vibrational temperature that efficiently transforms the vibrational energy into radiant energy, i.e. radiative heat flux. The whole process takes place in less than a millisecond which makes modeling a challenge. The experimental measurement of radiative heat flux (typically done with shock tubes) along with theoretical calculation through the unsteady Schrödinger equation are among the more esoteric aspects of aerospace engineering. Most of the aerospace research work related to understanding radiative heat flux was done in the 1960s but largely discontinued after conclusion of the Apollo Program. Radiative heat flux in air was just sufficiently understood to insure Apollo's success. However radiative heat flux in carbon dioxide (Mars entry) is still barely understood and will require major research.
Frozen gas model
The frozen gas model describes a special case of a gas that is not in equilibrium. The name "frozen gas" is misleading. A frozen gas is not "frozen" like ice is frozen water. Rather a frozen gas is "frozen" in time (all chemical reactions are assumed to have stopped). Chemical reactions are normally driven by collisions between molecules. If gas pressure is slowly reduced such that chemical reactions can continue then the gas can remain in equilibrium. However it is possible for gas pressure to be so suddenly reduced that almost all chemical reactions stop. For that situation the gas is considered frozen.
The distinction between equilibrium and frozen is important because it is possible for a gas such as air to have significantly different properties (speed-of-sound, viscosity, etc.) for the same thermodynamic state, e.g. pressure and temperature. Frozen gas can be a significant issue in the wake behind an entry vehicle. During reentry, free stream air is compressed to high temperature and pressure by the entry vehicle's shock wave. Non-equilibrium air in the shock layer is then transported past the entry vehicle's leading side into a region of rapidly expanding flow that causes freezing. The frozen air can then be entrained into a trailing vortex behind the entry vehicle. Correctly modeling the flow in the wake of an entry vehicle is very difficult. TPS heating in the vehicle's afterbody is usually not very high but the geometry and unsteadiness of the vehicle's wake can significantly influence aerodynamics (pitching moment) and particularly dynamic stability.
Thermal protection systems
Ablative
The type of heat shield that best protects against high heat flux is the ablative heat shield. The ablative heat shield functions by lifting the hot shock layer gas away from the heat shield's outer wall (creating a cooler boundary layer) through blowing. The overall process of reducing the heat flux experienced by the heat shield's outer wall is called blockage. Ablation causes the TPS layer to char, melt, and sublimate through the process of pyrolysis. The gas produced by pyrolysis is what drives blowing and causes blockage of convective and catalytic heat flux. Pyrolysis can be measured in real time using thermogravimetric analysis, so that the ablative performance can be evaluated.[8] Ablation can also provide blockage against radiative heat flux by introducing carbon into the shock layer thus making it optically opaque. Radiative heat flux blockage was the primary thermal protection mechanism of the Galileo Probe TPS material (carbon phenolic). Carbon phenolic was originally developed as a rocket nozzle throat material (used in the Space Shuttle Solid Rocket Booster) and for RV nose tips. Thermal protection can also be enhanced in some TPS materials through coking. Coking is the process of forming solid carbon on the outer char layer of the TPS. TPS coking was discovered accidentally during development of the Apollo-CM TPS material (Avcoat 5026-39).
The thermal conductivity of a TPS material is proportional to the material's density. Carbon phenolic is a very effective ablative material but also has high density which is undesirable. If the heat flux experienced by an entry vehicle is insufficient to cause pyrolysis then the TPS material's conductivity could allow heat flux conduction into the TPS bondline material thus leading to TPS failure. Consequently for entry trajectories causing lower heat flux, carbon phenolic is sometimes inappropriate and lower density TPS materials such as the following examples can be better design choices:
SLA-561V
"SLA" in SLA-561V stands for "Super Light weight Ablator". SLA-561V is a proprietary ablative made by Lockheed Martin that has been used as the primary TPS material on all of the 70 degree sphere-cone entry vehicles sent by NASA to Mars. SLA-561V begins significant ablation at a heat flux of approximately 75 W/cm² but will fail for heat fluxes greater than 300 W/cm². The Mars Science Laboratory (MSL) aeroshell TPS is currently designed to withstand a peak heat flux of 234 W/cm². The peak heat flux experienced by the Viking-1 aeroshell which landed on Mars was 21 W/cm². For Viking-1, the TPS acted as a pure thermal insulator and never experienced significant ablation. Viking-1 was the first Mars lander and based upon a very conservative design. The Viking aeroshell had a base diameter of 3.54 meters (the largest yet used on Mars). SLA-561V is applied by packing the ablative material into a honeycomb core that is pre-bonded to the aeroshell's structure thus enabling construction of a large heat shield.
PICA
Phenolic Impregnated Carbon Ablator (PICA) was developed by NASA Ames Research Center and was the primary TPS material for the Stardust aeroshell[9]. Because the Stardust sample-return capsule was the fastest man-made object to reenter Earth's atmosphere (~12.4 km/sec / ~28,000 mph relative velocity at 135 km altitude), PICA was an enabling technology for the Stardust mission. (For reference, the Stardust reentry was faster than the Apollo Mission capsules and 70% faster than the reentry velocity of the Shuttle.) PICA is a modern TPS material and has the advantages of low density (much lighter than carbon phenolic) coupled with efficient ablative capability at high heat flux. Stardust's heat shield (0.81 m base diameter) was manufactured from a single monolithic piece sized to withstand a nominal peak heating rate of 1200 W/cm2. PICA is a good choice for ablative applications such as high-peak-heating conditions found on sample-return missions or lunar-return missions. PICA's thermal conductivity is lower than other high-heat-flux ablative materials, such as conventional carbon phenolics.
SIRCA
Silicone Impregnated Reuseable Ceramic Ablator (SIRCA) was also developed at NASA Ames Research Center and was used on the Backshell Interface Plate (BIP) of the Mars Pathfinder and Mars Exploration Rover (MER) aeroshells. The BIP was at the attachment points between the aeroshell's backshell (also called the "afterbody" or "aft cover") and the cruise ring (also called the "cruise stage"). SIRCA was also the primary TPS material for the unsuccessful Deep Space 2 (DS/2) Mars probes with their 0.35 m base diameter aeroshells. SIRCA is a monolithic, insulative material that can provide thermal protection through ablation. It is the only TPS material that can be machined to custom shapes and then applied directly to the spacecraft. There is no post-processing, heat treating, or additional coatings required (unlike current Space Shuttle tiles). Since SIRCA can be machined to precise shapes, it can be applied as tiles, leading edge sections, full nose caps, or in any number of custom shapes or sizes. SIRCA has been demonstrated in BIP applications but not yet as a forebody TPS material.[10]
Early research on ablation technology in the USA was centered at NASA's Ames Research Center located at Moffett Field, California. Ames Research Center was ideal, since it had numerous wind tunnels capable of generating varying wind velocities. Initial experiments typically mounted a mock-up of the ablative material to be analyzed within a hypersonic wind tunnel.[11]
Thermal soak
Thermal soak is a part of almost all TPS schemes. For example, an ablative heat shield loses most of its thermal protection effectiveness when the outer wall temperature drops below the minimum necessary for pyrolysis. From that time to the end of the heat pulse, heat from the shock layer soaks into the heat shield's outer wall and would eventually convect to the payload. This outcome is prevented by ejecting the heat shield (with its heat soak) prior to the heat convecting to the inner wall.
Thermal soak TPS is intended to shield mainly against heat load and not against a high peak heat flux (a long duration heat pulse of low intensity is assumed for the TPS design). The Space Shuttle orbit vehicle was designed with a reusable heat shield based upon a thermal soak TPS. It should be emphasized that the tradeoff for TPS reusability is an inability to withstand a high heat flux, e.g. a Space Shuttle TPS would not be practical as a primary thermal protection for lunar return. A Space Shuttle's underside is coated with thousands of tiles made of silica foam, which are intended to survive multiple reentries with only minor repairs between missions. Fabric sheets known as gap fillers are inserted between the tiles where necessary. These gap fillers provide for a snug fit between separate tiles while allowing for thermal expansion. When a Space Shuttle lands, a significant amount of heat is stored in the TPS. Shortly after landing, a ground-support cooling unit connects to the Space Shuttle's internal Freon coolant loop to remove heat soaked in the TPS and orbiter structure.
Typical Space Shuttle's TPS tiles (LI-900) have remarkable thermal protection properties but are relatively brittle and break easily. An LI-900 tile exposed to a temperature of 1000 K on one side will remain merely warm to the touch on the other side. An impressive stunt that can be performed with a cube of LI-900 is to remove it glowing white hot from a furnace and then hold it with one's bare fingers without discomfort along the cube's edges.
Passively cooled
In some early ballistic missile RVs, e.g. the Mk-2 and the sub-orbital Mercury spacecraft, radiatively cooled TPS were used to initially absorb heat flux during the heat pulse and then after the heat pulse, radiate and convect the stored heat back into the atmosphere. Unfortunately, the earlier version of this technique required a considerable quantity of metal TPS (e.g. titanium, beryllium, copper, etc.), adding greatly to the vehicle's mass. Consequently ablative and thermal soak TPS have become preferable.
Radiatively cooled TPS can still be found on modern entry vehicles but Reinforced Carbon-Carbon (also called RCC or carbon-carbon) is normally used instead of metal. RCC is the TPS material on the leading edges of the Space Shuttle's wings. RCC was also proposed as the leading edge material for the X-33. Carbon is the most refractory material known with a one atmosphere sublimation temperature of 3825 °C for graphite. This high temperature made carbon an obvious choice as a radiatively cooled TPS material. Disadvantages of RCC are that it is currently very expensive to manufacture and lacks impact resistance.
Some high-velocity aircraft, such as the SR-71 Blackbird and Concorde, had to deal with heating similar to that experienced by spacecraft but at much lower intensity. Studies of the SR-71's titanium skin revealed the metal structure was restored to its original strength through annealing due to aerodynamic heating. In the case of Concorde the aluminium nose was permitted to reach a maximum operating temperature of 127 °C (typically 180 °C warmer than the sub-zero ambient air); the metallurgical implications associated with the peak temperature was a significant factor determining the top speed of the aircraft.
A radiatively cooled TPS for an entry vehicle is often called a "hot metal TPS". Early TPS designs for the Space Shuttle called for a hot metal TPS based upon titanium shingles. Unfortunately the earlier Shuttle TPS concept was rejected because it was incorrectly believed a silica tile based TPS offered less expensive development and manufacturing costs. A titanium shingle TPS was again proposed for the unsuccessful X-33 Single-Stage to Orbit (SSTO) prototype.
Recently, newer radiatively cooled TPS materials have been developed that could be superior to RCC. Referred to by their prototype vehicle "SHARP" (Slender Hypervelocity Aerothermodynamic Research Probe), these TPS materials have been based upon substances such as zirconium diboride and hafnium diboride. SHARP TPS have suggested performance improvements allowing for sustained Mach 7 flight at sea level, Mach 11 flight at 100,000 ft altitudes and significant improvements for vehicles designed for continuous hypersonic flight. SHARP TPS materials enable sharp leading edges and nose cones to greatly reduce drag for air breathing combined cycle propelled space planes and lifting bodies. SHARP materials have exhibited effective TPS characteristics from zero to more than 2000 °C, with melting points over 3500 °C . They are structurally stronger than RCC thus not requiring structural reinforcement with materials such as Inconel. SHARP materials are extremely efficient at re-radiating absorbed heat thus eliminating the need for additional TPS behind and between SHARP materials and conventional vehicle structure. NASA initially funded (and discontinued) a multi-phase R&D program through the University of Montana in 2001 to test SHARP materials on test vehicles.[12][13]
Actively cooled
Various advanced reusable spacecraft and hypersonic aircraft designs have been proposed to employ heat shields made from temperature-resistant metal alloys that incorporated a refrigerant or cryogenic fuel circulating through them. Such a TPS concept was proposed for the X-30 National Aerospace Plane (NASP). The NASP was supposed to have been a scramjet powered hypersonic aircraft but failed in development.
In the early 1960s various TPS systems were proposed to use water or other cooling liquid sprayed into the shock layer. Such concepts never got past the proposal phase since ordinary ablative TPS is much more reliable and efficient.
Entry vehicle design considerations
There are four critical parameters considered when designing a vehicle for atmospheric entry:
- Peak heat flux
- Heat load
- Peak deceleration
- Peak dynamic pressure
Peak heat flux and dynamic pressure selects the TPS material. Heat load selects the thickness of the TPS material stack. Peak deceleration is of major importance for manned missions. The upper limit for manned return to Earth from Low Earth Orbit (LEO) or lunar return is 10 Gs. For Martian atmospheric entry after long exposure to zero gravity, the upper limit is 4 Gs. Peak dynamic pressure can also influence the selection of the outermost TPS material if spallation is an issue.
Starting from the principle of conservative design, the engineer typically considers two worst case trajectories, the undershoot and overshoot trajectories. The overshoot trajectory is typically defined as the shallowest allowable entry velocity angle prior to atmospheric skip-off. The overshoot trajectory has the highest heat load and sets the TPS thickness. The undershoot trajectory is defined by the steepest allowable trajectory. For manned missions the steepest entry angle is limited by the peak deceleration. The undershoot trajectory also has the highest peak heat flux and dynamic pressure. Consequently the undershoot trajectory is the basis for selecting the TPS material. There is no "one size fits all" TPS material. A TPS material that is ideal for high heat flux may be too conductive (too dense) for a long duration heat load. A low density TPS material might lack the tensile strength to resist spallation if the dynamic pressure is too high. A TPS material can perform well for a specific peak heat flux but fail catastrophically for the same peak heat flux if the wall pressure is significantly increased (this happened with NASA's R-4 test spacecraft).[14] Older TPS materials tend to be more labor intensive and expensive to manufacture compared to modern materials. However modern TPS materials often lack the flight history of the older materials (an important consideration for a risk adverse designer).
Based upon Allen and Eggers discovery, maximum aeroshell bluntness (maximum drag) yields minimum TPS mass. Maximum bluntness (minimum ballistic coefficient) also yields a minimal terminal velocity at maximum altitude (very important for Mars EDL but detrimental for military RVs). However there is an upper limit to bluntness imposed by aerodynamic stability considerations based upon shock wave detachment. A shock wave will remain attached to the tip of a sharp cone if the cone's half-angle is below a critical value. This critical half-angle can be estimated using perfect gas theory (this specific aerodynamic instability occurs below hypersonic speeds). For a nitrogen atmosphere (Earth or Titan), the maximum allowed half-angle is approximately 60°. For a carbon dioxide atmosphere (Mars or Venus), the maximum allowed half-angle is approximately 70°. After shock wave detachment, an entry vehicle must carry significantly more shocklayer gas around the leading edge stagnation point (the subsonic cap). Consequently, the aerodynamic center moves upstream thus causing aerodynamic instability. It is incorrect to reapply an aeroshell design intended for Titan entry (Huygens probe in a nitrogen atmosphere) for Mars entry (Beagle-2 in a carbon dioxide atmosphere). After being abandoned, the Soviet Mars lander program achieved no successful landings (no useful data returned) after multiple attempts. The Soviet Mars landers were based upon a 60° half-angle aeroshell design. In the early 1960s, it was incorrectly believed the Martian atmosphere was mostly nitrogen, (actual Martian atmospheric mole fractions are carbon dioxide 0.9550, nitrogen 0.0270 and argon 0.0160). The Soviet aeroshells were probably(?) based upon an incorrect Martian atmospheric model and then not revised when new data became available.
A 45 degree half-angle sphere-cone is typically used for atmospheric probes (surface landing not intended) even though TPS mass is not minimized. The rationale for a 45° half-angle is either aerodynamic stability from entry-to-impact (the heat shield is not jettisoned) or a short-and-sharp heat pulse followed by prompt heat shield jettison. A 45° sphere-cone design was used with the DS/2 Mars landers and Pioneer Venus Probes.
History's most difficult atmospheric entry
The highest speed controlled entry so far achieved was by the Galileo Probe. The Galileo Probe was a 45° sphere-cone that entered Jupiter's atmosphere at 47.4 km/s (atmosphere relative speed at 450 km above the 1 bar reference altitude). The peak deceleration experienced was 230 Gs. Peak stagnation point pressure before aeroshell jettison was 9 bars. The peak shock layer temperature was approximately 16000 K (the solar photosphere is merely 5800 K). Approximately 26% of the Galileo Probe's original entry mass of 338.93 kg was vaporized during the 70 second heat pulse. Total blocked heat flux peaked at approximately 15000 W/cm². By way of comparison, the peak total heat flux experienced by the Mars Pathfinder aeroshell was 106 W/cm² (highest experienced by a successful Mars lander). The Apollo-4 (AS-501) command module which reentered the Earth's atmosphere at a velocity of 10.77 km/s (atmosphere relative speed at 121.9 km altitude) experienced a peak total heat flux of 497 W/cm².
Conservative design was used in creating the Galileo Probe. Due to the extreme state of the Galileo Probe's entry conditions, the radiative heat flux and turbulence of the shock layer along with the TPS material response were barely understood. Carbon Phenolic was used for the Galileo Probe TPS. Carbon phenolic was earlier used for the Pioneer Venus Probes which were the design ancestors to the Galileo Probe. The Galileo Probe experienced far greater TPS recession near the base of its frustum than expected. Despite a factor of two safety-factor in TPS thickness, the Galileo Probe's heatshield almost failed. The precise mechanism for this higher TPS recession is still unknown and currently beyond definitive theoretical analysis.
After successfully completing its mission, the Galileo Probe continued descending into Jupiter's atmosphere where the ambient temperature grew with greater depth due to isentropic compression. In the unfathomable depths of Jupiter's atmosphere, the surrounding temperature became so hot that the entire probe including its jettisoned heat shield vaporized into monatomic gas.
Notable atmospheric entry mishaps
Not all atmospheric re-entries have been successful and some have led to significant disasters.
- Vostok 1 – The service module failed to detach for 10 minutes, but fortunately, Cosmonaut Yuri Gagarin survived.
- Mercury 6 – Instrument readings show that the heat shield and landing bag were not locked. The decision was made to leave the retrorocket pack in position during reentry. Astronaut John Glenn survived. The instrument readings were later found to be erroneous.
- Voskhod 2 – The service module failed to detach for some time, but the crew survived.
- Soyuz 1 – Different accounts exist. Either the attitude control system failed while still in orbit and/or parachutes got entangled during the landing sequence (entry, descent and landing (EDL) failure). Cosmonaut Vladimir Mikhailovich Komarov died.
- Soyuz 5 – The service module failed to detach, but crew survived.
- Soyuz 11 – The crew perished due to early depressurization.
- Mars Polar Lander (MPL) – Failed during EDL. The failure was believed to be the consequence of a software error. The precise cause is unknown due to lack of real time telemetry.
- Space Shuttle Columbia – The failure of an RCC tile on a wing leading edge led to breakup of the orbit vehicle at hypersonic speed resulting in the loss of all seven crew members. This is probably the most infamous incident.
- Genesis – The parachute failed to deploy due to a G-switch being installed backwards (a similar error delayed parachute deployment for the Galileo Probe). Consequently, the Genesis entry vehicle augered into the desert floor. The payload was damaged but it was later claimed that some scientific data was recoverable.
Uncontrolled reentry
More than 100 metric tons of man-made objects reenter in an uncontrolled fashion each year. The vast majority burn up before reaching earth's surface. On average, about one cataloged object reenters per day. Approximately a quarter of all objects are of U.S. origin. Due to the Earth's surface being primarily water, most objects that survive reentry land in one of the world's oceans.
In 1978, Cosmos 954 reentered uncontrolled and crashed near Great Slave Lake in the Northwest Territories of Canada. Cosmos 954 was nuclear powered, using a nuclear fission reactor, and spread radioactive debris across northern Canada.
In 1979, Skylab reentered uncontrolled and crashed into Western Australia, killing one cow and damaging several buildings. Australia issued a fine for littering to the United States, but the fine was never settled.[15]
Deorbit disposal
In 2001, the Russian Mir space station was deliberately de-orbited, and broke apart during atmospheric re-entry. Mir entered the Earth's atmosphere on March 23, 2001, near Nadi, Fiji, and fell into the South Pacific Ocean.
Research into atmospheric entry
As with many technologies, aerospace technological information can be dual use, i.e. aerospace technology can be used for both civilian or military purpose. Atmospheric entry technology owes its origins to the development of ballistic missiles during the Cold War. Given the enormous expense required in developing this technology, it is doubtful it could have appeared as quickly as it did without the military incentive. Mankind's survival beyond its planet of origin could be dependent upon atmospheric entry technology. It is ironic that the same technology enabling destructive nuclear-tipped missiles also enables this exploration and development of outer space. Aerospace technology is needed for civilian space exploration, yet certain aspects are and will remain restricted to impede military proliferation of the technology. This basic dilemma is present throughout the literature on atmospheric entry. There is a glass wall between pedagogical and practical information. For example, in the text books referenced in this article, a topic thread will proceed as long as the information is nonspecific but almost always stops at the point of practical application. To go beyond pedagogical information, one must search the technical literature (NACA/NASA Technical Reports, declassified technical reports and peer reviewed archive literature). Declassified technical reports are a frustrating information source since many of the reports were destroyed prior to going through the legally required declassification process. It is almost always true that significant documents referred to in declassified technical reports no longer exist (technical information costing many millions of dollars has simply vanished).
Warheads
When the reentry vehicle is a guided missile, intended to cause a destructive effect at the end of its reentry path, it is called a warhead. This may be a nuclear weapon, or, when the reentry vehicle is a precision-guided munition, an inert filler such as concrete or steel. Inert fillers in reentry vehicles have military significance because the kinetic energy of a reentry vehicle is so great that adding chemical explosives to the warhead would only contribute insignificantly to the energy release.
The part of the missile that carries a warhead may also carry multiple warheads. A multiple warhead carrier assembly is called a bus. A multiple reentry vehicle (MRV) carrier releases all its warheads at the same time, but causes them to spread apart as they reenter, perhaps with a spring or a small explosive charge.
If the bus releases the warheads at different times in its flight, perhaps with a mechanical positioner on the warhead, or rocket controls that reorient the bus, each warhead is part of a set called a multiple independently targeted reentry vehicle (MIRV). Once released by the bus, the basic MIRV warheads follow strictly ballistic trajectories.
Individual reentry vehicles that use rockets, shifting center of mass, or aerodynamic control to alter their course from the purely ballistic course belong to the class of maneuverable reentry vehicles (MARV).
MIRV and MARV systems present especially difficult problems in arms control, because while a reconnaissance satellite can verify a land-based ICBM is in its silo, the sensors cannot look inside the aerodynamic shroud around the nose of the missile and see how many reentry vehicles, of what type, are inside.
A MIRV or MARV capable bus may also release penetration aids to make it more difficult to track or intercept the real warheads. Penetration aids are a highly classified technology, and the criteria by which a sensor can discriminate a penetration aid from a warhead are usually among the most highly classified parts of a missile system. Nevertheless, it is known that inflatable balloons covered with a thin metal film are used as one kind of radar reflector.
Since the balloon will have more air resistance than a solid warhead, it will soon reveal itself by taking a different trajectory. There may be more solid penetration aids that are made up of angles intended to cause maximum radar reflection, the antithesis of a stealth aircraft or weapon. Other techniques may exist, such as active radar transmitters that can overload or deceive missile defense radar.
References
- ↑ Allen, H. Julian and Eggers, Jr., A. J., "A Study of the Motion and Aerodynamic Heating of Ballistic Missiles Entering the Earth's Atmosphere at High Supersonic Speeds," NACA Report 1381, (1958).
- ↑ Fay, J. A. and Riddell, F. R., "Theory of Stagnation Point Heat Transfer in Dissociated Air," Journal of the Aeronautical Sciences, Vol. 25, No. 2, page 73, February 1958 (see "Fay-Riddell equation" entry in Glossary of atmospheric reentry).
- ↑ Hillje, Ernest R., "Entry Aerodynamics at Lunar Return Conditions Obtained from the Flight of Apollo 4 (AS-501)," NASA TN D-5399, (1969).
- ↑ Regan, Frank J. and Anadakrishnan, Satya M., "Dynamics of Atmospheric Re-Entry," AIAA Education Series, American Institute of Aeronautics and Astronautics, Inc., New York, ISBN 1-56347-048-9, (1993).
- ↑ [1]Ames Research Staff, "Equations, Tables, and Charts for Compressible Flow," NACA Report 1135, (1953).]
- ↑ Lighthill, M.J., "Dynamics of a Dissociating Gas. Part I. Equilibrium Flow," Journal of Fluid Mechanics, vol. 2, pt. 1. p. 1 (1957).
- ↑ Freeman, N.C., "Non-equilibrium Flow of an Ideal Dissociating Gas." Journal of Fluid Mechanics, vol. 4, pt. 4, p. 407 (1958).
- ↑ Parker, John and C. Michael Hogan, "Techniques for Wind Tunnel assessment of Ablative Materials," NASA Ames Research Center, Technical Publication, August, 1965.
- ↑ Tran, Huy K, et al., "Qualification of the forebody heatshield of the Stardust's Sample Return Capsule," AIAA, Thermophysics Conference, 32nd, Atlanta, GA; 23-25 June 1997.
- ↑ Tran, Huy K., et al., "Silicone impregnated reusable ceramic ablators for Mars follow-on missions," AIAA-1996-1819, Thermophysics Conference, 31st, New Orleans, LA, June 17-20, 1996.
- ↑ Hogan, C. Michael, Parker, John and Winkler, Ernest, of NASA Ames Research Center, "An Analytical Method for Obtaining the Thermogravimetric Kinetics of Char-forming Ablative Materials from Thermogravimetric Measurements", AIAA/ASME Seventh Structures and Materials Conference, April, 1966
- ↑ http://hubbard.engr.scu.edu/docs/thesis/2003/SHARP_Thesis.pdf
- ↑ http://www.coe.montana.edu/me/faculty/cairns/sharp/sharp.htm
- ↑ Pavlosky, James E., St. Leger, Leslie G., "Apollo Experience Report - Thermal Protection Subsystem," NASA TN D-7564, (1974).
- ↑ Australians Take Mir Deorbit Risks in Stride, Space.com